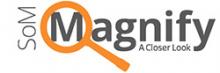
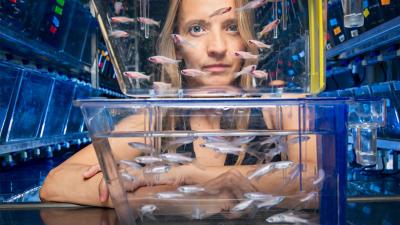
A Small Fish in a Big Question
The questions pursued by Eva Naumann’s research team are nearly as large and intricate as the brain itself: How does the entire brain transform sensory inputs into actions, and what neural pathways do these impulses travel?
Even in the relatively small brain of the 3-millimeter translucent larval zebrafish she studies, it’s a question that involves ridiculous levels of complexity. For comparison, our human brain contains 86 billion neurons, whereas the zebrafish contains a manageable 100,000. Naumann’s goal is to map the ways these neurons functionally connect to each other to produce thoughts and behavior in this relatively tiny vertebrate brain.
“I’m not afraid of complexity,” Naumann says from behind a colorful mask, sitting in her sunny but spare Bryan Research office. “That’s probably what defines my approach to disentangling the many functions of neural circuits in these little brains. Sometimes it can be dangerous.”
Fortunately for her, technology marches forward, and the tools she needs to see the nervous system’s complexity and interpret its meaning have been growing according to Moore’s Law. Advances in microscopy, genetically engineered fluorescent activity sensors, and optogenetic actuators now permit the visualization and manipulation of all neurons in the zebrafish brain.
“None of these tools are unique to zebrafish. I just think the zebrafish might be the first vertebrate model system where you can actually map every single neuron, and possibly, in the future, every single synapse, and build a quanitative model of the entire brain,” said Naumann, an assistant professor of neurobiology in the School of Medicine. “You could possibly, especially with modern computational methods, map out the information flow across brain-scale neural circuits computing diverse information.”
It’s something Naumann has done already, in a study of “optomotor response,” the connection between what a fish sees and how it twitches its tail in response. A 2016 paper in Cell resulted in a map of the fish brain showing detailed activation patterns from interconnected neurons in the retina to those sending commands to the fish’s tail muscles.
Somewhat surprisingly, there’s no single element of the circuit that interprets the data alone. Rather, the entire network collaborates to combine, compare, and filter the information it receives from both eyes. The activity patterns created by the involved neurons essentially distribute the sensory information and transform it into executable motor responses.
Naumann wanted to know the minimum number of neurons required to build such a circuit. Working with Harvard colleague James Fitzgerald for about six months, she was determined to find the minimal model that could explain the behavior.
“To our surprise, the solution was, theoretically, you might not need any interpreter! Either you need a linear or nonlinear transfer function for that behavior that we described. And you really don't need anything else. It was kind of a bummer answer,” Naumann said, because it meant the circuit could be simpler than was suggested by the many activated neurons she observed but raised important questions about what all the motion activated neurons were doing. To explain their function, the scientists used a biologically constrained “neural network” computer model of the zebrafish brain to arrive at an answer.
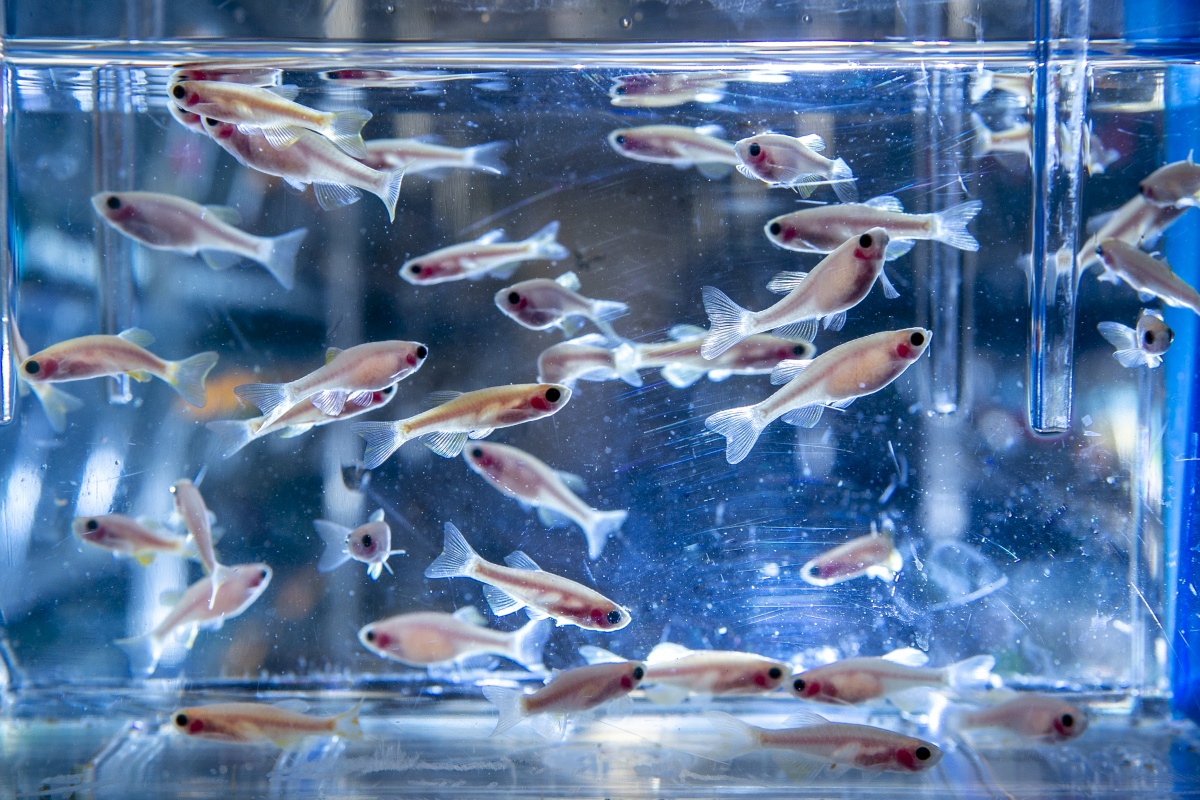
A Beautiful Balance
When Naumann came to Harvard from Germany for her PhD, which she received in 2010, the usual approach to behavioral data collection was “Hire an undergrad to watch movies to score behavioral events.” To try to automate the process, Naumann took it upon herself to learn about computer vision — the ability of tireless, unblinking machines to “see” and code behavior. “I was a terrible programmer, because that really wasn’t my background,” she said, but it gave her a start at using machines to observe behavior at time-scales and complexity that lie well beyond human ability.
The basic setup is simple: A live, untethered fish in a dish of water, and a small projector beneath it showing a moving pattern of stripes that create the impression of motion. The neural response to the illusion of being sent adrift happens within milliseconds, and baby fish swim fast enough when their brain says “Move now!” that it’s necessary to capture them at around 200 frames per second.
“Initially, I think, it sounds so boring to ask why a fish swims left,” Naumann said. “But the more you think about it, it is actually quite complex. The optomotor response, or any basic motion-vision study, has this kind of beautiful balance of simplicity and complexity.”
“Initially, I think, it sounds so boring to ask why a fish swims left. But the more you think about it, it is actually quite complex. The optomotor response, or any basic motion-vision study, has this kind of beautiful balance of simplicity and complexity.” -- Eva Naumann
What she understands so far is that retinal neurons are dedicated information channels — encoding up, down, right, left etc. — and that the system relies in part on the interference between signals coming in from two different eyes.
“In the zebrafish, you really can map out exactly, for example, how this neuron cares about this type of motion from one eye and this type of motion from the other eye,” she said. “Some neurons add, and some neurons are suppressed by the other eye. It’s pretty interesting to probe what the nervous system cares about.”
Yes, it’s just a fish — a vertebrate like us, yet far more simple. But it’s Naumann’s window to a wider world.
She holds up her left hand in a fist. “If you look at my fist here, if this is the mouse brain or the mammalian brain, we can basically image it only skin deep. But the zebrafish brain is only skin-thick,” making all of it accessible, she explains.
The all-optical techniques Naumann’s group is using on fish are being applied to mammalian brains already, “it’s fantastic and the results on local circuit mapping are really cool. But what they can't do—because of the mammalian brain’s size— is to use these techniques to holistically map out the functional connectivity across the brain and see what effect they have in a different brain region, revealing how single neurons functionally connect to the rest of the brain. We are applying these powerful all-optical methods in the tiny zebrafish to do exactly this in the context of sensory processing.”
Building Connections
Naumann’s setup also includes a wet lab for editing light-sensitive proteins into the genes of fish and “the kitchen,” a room where new devices are built, often with the help of the lab’s 3D printer.
In a corner room with blacked-out windows, dominated by a cube of black draping, postdoctoral researcher Maxim Nikitchenko is using his background in physics to fine-tune an innovative, purpose-built microscope. Boxes of fasteners and hand tools are strewn across almost every surface.
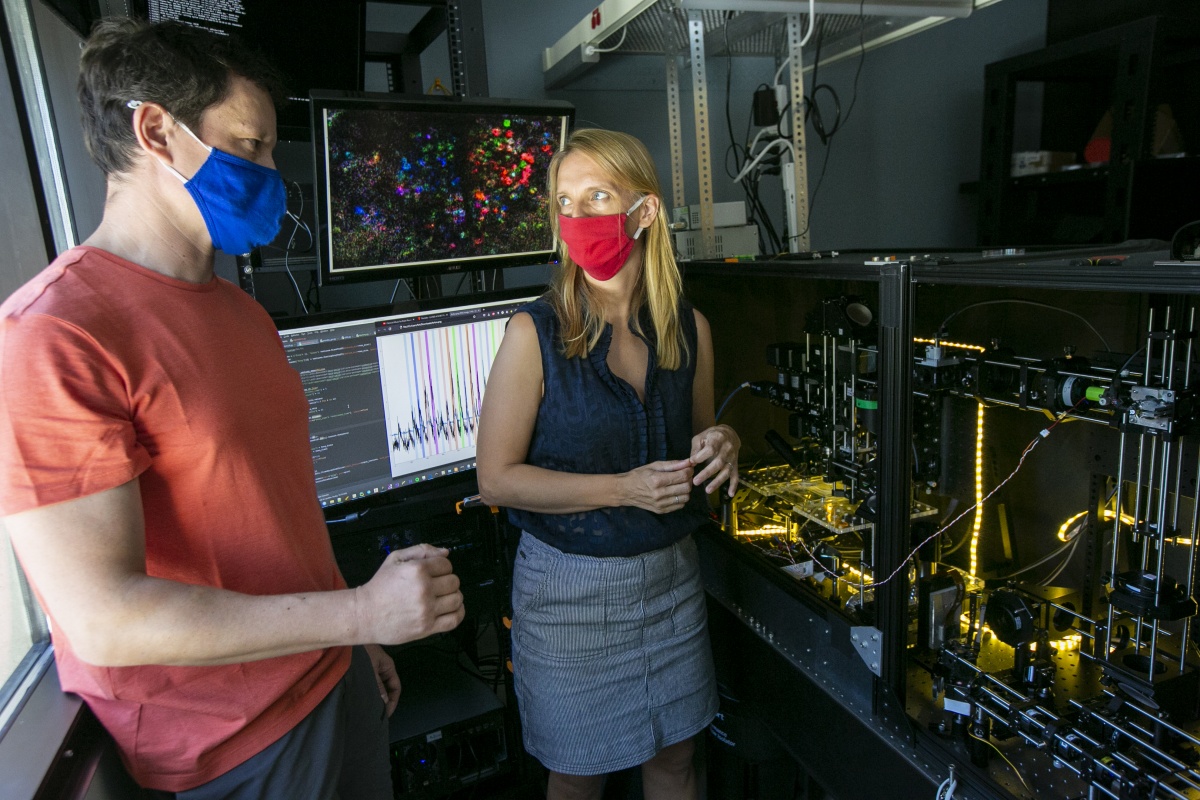
Two and a half years in the making (so far), the contraption uses a dual beam two-photon laser source to simultaneously stimulate and measure individual neurons in a living embryo.
“We're trying to build a method that is called all-optical recording because it allows us to 'read' and 'write' neural activity in the same experiment,” Naumann explains. “If you think that a neuron is interesting, you can shine this tuned red laser light onto the neuron and basically push on it and ask, ‘What do you connect to?’ What we’re trying to build is a system that gives you random access, at varying strengths, for each neuron.”
They hope to do this at a rate of a million points of light per second in multiple planes at once, because even simple brains work really, really fast. With holography, a 3D “army of light spots,” Naumann hopes to go beyond statistical correlations and actually visualize how one neuron connects to the others. To develop real-time analysis of neural activity (i.e., faster than acquisition rate), she teamed up with John Pearson in the Duke Biostatistics Department to classify neurons across the brain. Using this fast analysis they plan to design adaptive, interactive and automated photostimulation of neurons, testing causal relationships by measuring downstream activity after photostimulation.
And it’s not enough to see the neurons communicating with each other. She wants to listen in on their conversations too. She calls this “Functional Connectivity Mapping.”
Tracking Neural Responses
Duke Neurobiology is a great home for Naumann because many of its investigators are also system neuroscientists like her, she said. She joined Duke in 2018. Earlier this year, she received a prestigious Alfred P. Sloan Foundation two-year fellowship for being a pathbreaking young scientist.
The new imaging rig will allow Naumann’s group to expand beyond mapping motor responses to visual stimuli and begin mapping other things, such as response to smells, response to gut stimulation, and so on. Graduate student Minel Arinel is working on the gut project in collaboration with John Rawls’ lab in the Duke Microbiome Center.
Stimulating the gut is quite a bit trickier than just showing movies of stripes to the fish, so Arinel is developing a device to deliver tiny amounts of chemicals directly into a fish’s gut with a hair-thin needle, held steady by a custom-built 3D printed stand.
Arinel’s question is essentially the same: If the fish’s gut is wired to the brain, is it possible to see an impulse travel from stimulus to response and observe all the connections it made along its microsecond journey?
Nauman asks, “How is the patterned activity of these neurons giving rise to patterned activity that we observe in the fish?”
Sitting in front of an array of three computer screens, each of which is split into many panels of fish video, lines of code, and data plots, graduate student Matt Loring is writing and validating the code that turns all these split-second observations into interpretable data.
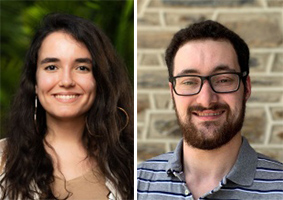
“He came from a molecular lab, but it turns out he’s even better at programming,” Naumann said of Loring.
“We can color code neurons according to what response they like,” Loring said. “In this case, blue is leftward responses and red is rightward responses. And we can pseudo-color neurons.”
Still, some interpretations may be easier said than done. For example, what if the fish has other things going on in its mind? “In our idealized world, the attention is always 100 percent, and the only thing they care about is the stimuli we show them,” Loring said. But that may not always be the case. And, just like people, no two fish are exactly alike.
“What we hope is that the automated analysis has an unbiased view of whether this is an active neuron or not,” Naumann said. “And then you can say, okay, we only choose neurons that are active. (Matt) really allowed the machine to learn what kind of neurons we see in our recordings.”
Since arriving at Duke, Naumann has worked with Roarke Horstmeyer’s lab in Duke biomedical engineering, which specializes in computational optics. Together, they’re developing a scalable multicamera array microscope with an unprecedentedly large field of view. She hopes that her collaborative work on these gigapixel recordings of behavior and neural activity in hundreds of zebrafish behaving naturally will offer insights into the long-term dynamics of behavior and brain function.
Limitations and Opportunities
There are some drawbacks to the larval fish model. One, as noted, they’re fish. And two, at that early age they don’t have a fully developed suite of fish behaviors. Only after about 21 days do they show social behaviors, learning, memory, fear, addiction, and other responses that would be more useful for interpreting humans. But by then, their skull starts to be covered by opaque bone, and thus harder to see through.
With that limitation in mind, Naumann is considering adding another kind of fish to her studies: Danionella translucida, a dwarf relative of the zebrafish from Myanmar that, amazingly, has an open patch at the top of its skull even in adulthood, giving neuroscientists a literal window into the mind.
Loring is also interested in understanding neuronal wiring at the very earliest stages of zebrafish development, before the escape response has developed. “There are a few days where it goes from not behaving at all, to behaving at intermediate levels, and then sort of a full suite of behaviors,” Loring said.
From six to 12 days, the developing zebrafish will double the number of neurons in their brains. But even at six days, they’re already capable of sensing motion and moving their tail in an appropriate response. So, why all the added neurons, and what are they doing?
“What happens early, versus those next six days?” Loring asks. “How stable is a neuron?”
“What Matt hopefully will find out is how the different neurons that have different qualities get added to the system,” Naumann said. “And I think he will be able to mess around with them to see why do you need to add this type of neuron?”
It may seem odd to devote all this attention to a pinprick of brain tissue in a tiny fish, but the tools and techniques the group is establishing and the deeper understanding of neural circuitry they will reveal create a foundation for subsequent investigation.
“Many neuropsychiatric diseases don’t only involve just one neuron type, or they affect not only one brain area but many areas,” Naumann said. “Maybe what we really need is circuit therapies for these complex diseases. This is why we need to understand better how different neurons talk to each other, and what may go wrong.”
At this point, we’re a long way from using the zebrafish to heal, for example, Alzheimer's disease.
“But hopefully, in maybe 30 years, I will be much closer to being able to explain to you how connectivity basically works,” Naumann said.
“Curiosity drives the best research,” she declared. “And to me it seems like, if you actually could understand the fish, you probably would have a much easier time understanding other models.”
Story by Karl Leif Bates, Executive Director of Research Communications at Duke University. Photos by Jared Lazarus, Senior Multimedia Producer at Duke University.